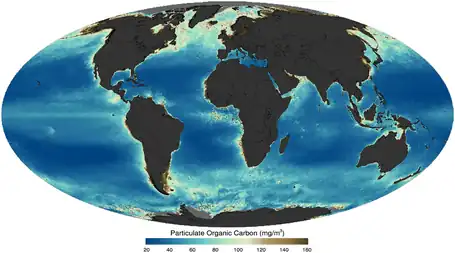
as imaged by satellite in 2011
The Martin curve is a power law used by oceanographers to describe the export to the ocean floor of particulate organic carbon (POC). The curve is controlled with two parameters: the reference depth in the water column, and a remineralisation parameter which is a measure of the rate at which the vertical flux of POC attenuates.[1] It is named after the American oceanographer John Martin.
The Martin Curve has been used in the study of ocean carbon cycling and has contributed to understanding the role of the ocean in regulating atmospheric CO2 levels.
Background
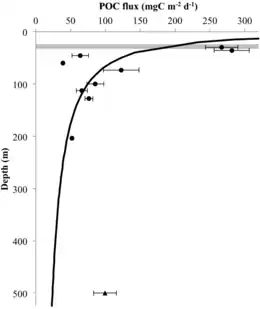
Part of a series on the |
Carbon cycle |
---|
![]() |
|
The dynamics of the particulate organic carbon (POC) pool in the ocean are central to the marine carbon cycle. POC is the link between surface primary production, the deep ocean, and marine sediments. The rate at which POC is degraded in the dark ocean can impact atmospheric CO2 concentration.[2]
The biological carbon pump (BCP) is a crucial mechanism by which atmospheric CO2 is taken up by the ocean and transported to the ocean interior. Without the BCP, the pre-industrial atmospheric CO2 concentration (~280 ppm) would have risen to ~460 ppm.[3] At present, the particulate organic carbon (POC) flux from the surface layer of the ocean to the ocean interior has been estimated to be 4–13 Pg-C year−1.[4] To evaluate the efficiency of the BCP, it is necessary to quantify the vertical attenuation of the POC flux with depth because the deeper that POC is transported, the longer the CO2 will be isolated from the atmosphere. Thus, an increase in the efficiency of the BCP has the potential to cause an increase of ocean carbon sequestration of atmospheric CO2 that would result in a negative feedback on global warming.[5] Different researchers have investigated the vertical attenuation of the POC flux since the 1980s.[6][7][8][9][5]
In 1987, Martin et al. proposed the following power law function to describe the POC flux attenuation:[10]
- (1)
where z is water depth (m), and Fz and F100 are the POC fluxes at depths of z metres and 100 metres respectively. Although other functions, such as an exponential curve, have also been proposed and validated,[11][12] this power law function, commonly known as the "Martin curve", has been used very frequently in discussions of the BCP. The exponent b in this equation has been used as an index of BCP efficiency: the larger the exponent b, the higher the vertical attenuation rate of the POC flux and the lower the BCP efficiency.[13] Moreover, numerical simulations have shown that a change in the value of b would significantly change the atmospheric CO2 concentration.[14][15][16][5]
Subsequently, other researchers have derived alternative remineralization profiles from assumptions about particle degradability and sinking speed.[17][18][12][19][20][21][22][6] However, the Martin curve has become ubiquitous as the model that assumes slower-sinking and/or labile organic matter is preferentially depleted near the surface causing increasing sinking speed and/or remineralization timescale with depth.[10][23]
The Martin curve can be expressed in a slightly more general way as:[24]
where fp(z) is the fraction of the flux of particulate organic matter from a productive layer near the surface [25] sinking through the depth horizon z [m], Cp [mb] is a scaling coefficient, and b is a nondimensional exponent controlling how fp decreases with depth. The equation is often normalised to a reference depth zo but this parameter can be readily absorbed into Cp.[24]
Vertical attenuation rate
The vertical attenuation rate of the POC flux is very dependent on the sinking velocity and decomposition rate of POC in the water column. Because POC is labile and has little negative buoyancy, it must be aggregated with relatively heavy materials called ballast to settle gravitationally in the ocean. Materials that may serve as ballast include biogenic opal (hereinafter "opal"), CaCO3, and aluminosilicates. In 1993, Ittekkot hypothesized that the drastic decrease from ~280 to ~200 ppm of atmospheric CO2 that occurred during the last glacial maximum was caused by an increase of the input of aeolian dust (aluminosilicate ballast) to the ocean, which strengthened the BCP.[26] In 2002, Klaas and Archer , as well as Francois et al. who compiled and analyzed global sediment trap data, suggested that CaCO3, which has the largest density among possible ballast minerals, is globally the most important and effective facilitator of vertical POC transport, because the transfer efficiency (the ratio of the POC flux in the deep sea to that at the bottom of the surface mixed layer) is higher in subtropical and tropical areas where CaCO3 is a major component of marine snow.[27][28][5]
Reported sinking velocities of CaCO3-rich particles are high.[29][30] Numerical simulations that take into account these findings have indicated that future ocean acidification will reduce the efficiency of the BCP by decreasing ocean calcification.[31] In addition, the POC export ratio (the ratio of the POC flux from an upper layer (a fixed depth such as 100 metres, or the euphotic zone or mixed layer) to net primary productivity) in subtropical and tropical areas is low because high temperatures in the upper layer increase POC decomposition rates.[32] The result might be a higher transfer efficiency and a strong positive correlation between POC and CaCO3 in these low-latitude areas: labile POC, which is fresher and easier for microbes to break down, decomposes in the upper layer, and relatively refractory POC is transported to the ocean interior in low-latitude areas.[28][33][5]
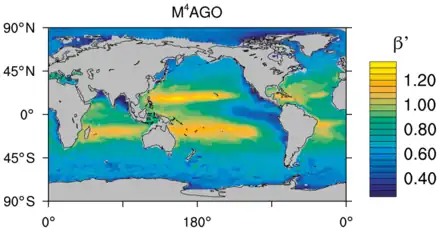
On the basis of observations that revealed a large increase of POC fluxes in high-latitude areas during diatom blooms and on the fact that diatoms are much bigger than coccolithophores,[35][36][37] Honda and Watanabe proposed in 2010 that opal, rather than CaCO3, is crucial as ballast for effective POC vertical transport in subarctic regions.[38] Weber et al. reported in 2016 a strong negative correlation between transfer efficiency and the picoplankton fraction of plankton as well as higher transfer efficiencies in high-latitude areas, where large phytoplankton such as diatoms predominate.[39] They also calculated that the fraction of vertically transported CO2 that has been sequestered in the ocean interior for at least 100 years is higher in high-latitude (polar and subpolar) regions than in low-latitude regions.[39][5]
In contrast, Bach et al.conducted in 2019 a mesocosm experiment to study how the plankton community structure affected sinking velocities and reported that during more productive periods the sinking velocity of aggregated particles was not necessarily higher, because the aggregated particles produced then were very fluffy; rather, the settling velocity was higher when the phytoplankton were dominated by small cells.[40] In 2012, Henson et al. revisited the global sediment trap data and reported the POC flux is negatively correlated with the opal export flux and uncorrelated with the CaCO3 export flux.[33][5]
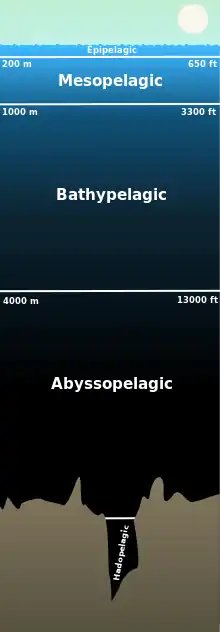
Key factors affecting the rate of biological decomposition of sinking POC in the water column are water temperature and the dissolved oxygen (DO) concentration: the lower the water temperature and the DO concentration, the slower the biological respiration rate and, consequently, the POC flux decomposition rate.[41][42][43][44] For example, in 2015 Marsay with other analysed POC flux data from neutrally buoyant sediment traps in the upper 500 m of the water column and found a significant positive correlation between the exponent b in equation (1) above and water temperature (i.e., the POC flux was attenuated more rapidly when the water was warmer).[41] In addition, Bach et al. found POC decomposition rates are high (low) when diatoms and Synechococcus (harmful algae) are the dominant phytoplankton because of increased (decreased) zooplankton abundance and the consequent increase (decrease) in grazing pressure.[40][5]
Using radiochemical observations (234Th-based POC flux observations), Pavia et al. found in 2019 that the exponent b of the Martin curve was significantly smaller in the low-oxygen (hypoxic) eastern Pacific equatorial zone than in other areas; that is, vertical attenuation of the POC flux was smaller in the hypoxic area.[44] They pointed out that a more hypoxic ocean in the future would lead to a lower attenuation of the POC flux and therefore increased BCP efficiency and could thereby be a negative feedback on global warming.[44] McDonnell et al. reported in 2015 that vertical transport of POC is more effective in the Antarctic, where the sinking velocity is higher and the biological respiration rate is lower than in the subtropical Atlantic.[45] Henson et al. also reported in 2019 a high export ratio during the early bloom period, when primary productivity is low, and a low export ratio during the late bloom period, when primary productivity is high.[46] They attributed the low export ratio during the late bloom to grazing pressure by microzooplankton and bacteria.[46][5]
Despite these many investigations of the BCP, the factors governing the vertical attenuation of POC flux are still under debate. Observations in subarctic regions have shown that the transfer efficiency between depths of 1000 and 2000 m is relatively low and that between the bottom of the euphotic zone and a depth of 1000 m it is relatively high.[41] Marsay et al. therefore proposed in 2015 that the Martin curve does not appropriately express the vertical attenuation of POC flux in all regions and that a different equation should instead be developed for each region.[41] Gloege et al. discussed in 2017 parameterization of the vertical attenuation of POC flux,[11] and reported that vertical attenuation of the POC flux in the twilight zone (from the base of the euphotic zone to 1000 m) can be parameterised well not only by a power law model (Martin curve) but also by an exponential model [18] and a ballast model.[17][5]
However, the exponential model tends to underestimate the POC flux in the midnight zone (depths greater than 1000 metres). Cael and Bisson reported in 2018 that the exponential model (power law model) tends to underestimate the POC flux in the upper layer, and overestimate it in the deep layer.[12] However, the abilities of both models to describe POC fluxes were comparable statistically when they were applied to the POC flux dataset from the eastern Pacific that was used to propose the "Martin curve".[10] In a long-term study in the northeastern Pacific, Smith et al. observed in 2018 a sudden increase of the POC flux accompanied by an unusually high transfer efficiency; they have suggested that because the Martin curve cannot express such a sudden increase, it may sometimes underestimate BCP strength.[47] In addition, contrary to previous findings, some studies have reported a significantly higher transfer efficiency, especially to the deep sea, in subtropical regions than in subarctic regions.[33][41][42] This pattern may be attributable to small temperature and DO concentration differences in the deep sea between high-latitude and low-latitude regions, as well as to a higher sinking velocity in subtropical regions, where CaCO3 is a major component of deep-sea marine snow. Moreover, it is also possible that POC is more refractory in low-latitude areas than in high-latitude areas.[33][41][42][5]
Uncertainty in the biological pump
The ocean's biological pump regulates atmospheric carbon dioxide levels and climate by transferring organic carbon produced at the surface by phytoplankton to the ocean interior via marine snow, where the organic carbon is consumed and respired by marine microorganisms. This surface to deep transport is usually described by a power law relationship of sinking particle concentration with depth. Uncertainty in biological pump strength can be related to different variable values (parametric uncertainty) or the underlying equations (structural uncertainty) that describe organic matter export. In 2021, Lauderdale evaluated structural uncertainty using an ocean biogeochemistry model by systematically substituting six alternative remineralisation profiles fit to a reference power-law curve. Structural uncertainty makes a substantial contribution, about one-third in atmospheric pCO2 terms, to the total uncertainty of the biological pump, highlighting the importance of improving biological pump characterisation from observations and its mechanistic inclusion in climate models.[24][48]
Carbon and nutrients are consumed by phytoplankton in the surface ocean during primary production, leading to a downward flux of organic matter. This "marine snow" is transformed, respired, and degraded by heterotrophic organisms in deeper waters, ultimately releasing those constituents back into dissolved inorganic form. Oceanic overturning and turbulent mixing return resource-rich deep waters back to the sunlit surface layer, sustaining global ocean productivity. The biological pump maintains this vertical gradient in nutrients through uptake, vertical transport, and remineralisation of organic matter, storing carbon in the deep ocean that is isolated from the atmosphere on centennial and millennial timescales, lowering atmospheric CO2 levels by several hundred microatmospheres.[49][50] The biological pump resists simple mechanistic characterisation due to the complex suite of biological, chemical, and physical processes involved,[51] so the fate of exported organic carbon is typically described using a depth-dependent profile to evaluate the degradation of sinking particulate matter.[24]
See also
References
- ↑ Olli, Kalle (2015). "Unraveling the uncertainty and error propagation in the vertical flux Martin curve". Progress in Oceanography. 135: 146–155. Bibcode:2015PrOce.135..146O. doi:10.1016/j.pocean.2015.05.016.
- ↑ Kharbush, J.J., Close, H.G., Van Mooy, B.A., Arnosti, C., Smittenberg, R.H., Le Moigne, F.A., Mollenhauer, G., Scholz-Böttcher, B., Obreht, I., Koch, B.P. and Becker, K. (2020) "Particulate Organic Carbon Deconstructed: Molecular and Chemical Composition of Particulate Organic Carbon in the Ocean". Frontiers in Marine Science, 7: 518. doi:10.3389/fmars.2020.00518.
Material was copied from this source, which is available under a Creative Commons Attribution 4.0 International License.
- ↑ Villa-Alfageme, M.; De Soto, F. C.; Ceballos, E.; Giering, S. L. C.; Le Moigne, F. A. C.; Henson, S.; Mas, J. L.; Sanders, R. J. (2016). "Geographical, seasonal, and depth variation in sinking particle speeds in the North Atlantic". Geophysical Research Letters. 43 (16): 8609–8616. Bibcode:2016GeoRL..43.8609V. doi:10.1002/2016GL069233. hdl:11441/98535. S2CID 3728545.
- ↑ Lima, I. D.; Lam, P. J.; Doney, S. C. (2014). "Dynamics of particulate organic carbon flux in a global ocean model". Biogeosciences. 11 (4): 1177–1198. Bibcode:2014BGeo...11.1177L. doi:10.5194/bg-11-1177-2014. hdl:1912/6674.
- 1 2 3 4 5 6 7 8 9 10 11 Honda, Makio C. (2020). "Effective Vertical Transport of Particulate Organic Carbon in the Western North Pacific Subarctic Region". Frontiers in Earth Science. 8: 366. Bibcode:2020FrEaS...8..366H. doi:10.3389/feart.2020.00366.
Material was copied from this source, which is available under a Creative Commons Attribution 4.0 International License.
- 1 2 Suess, Erwin (1980). "Particulate organic carbon flux in the oceans—surface productivity and oxygen utilization". Nature. 288 (5788): 260–263. Bibcode:1980Natur.288..260S. doi:10.1038/288260a0. S2CID 4275275.
- ↑ Betzer, Peter R.; Showers, William J.; Laws, Edward A.; Winn, Christopher D.; Ditullio, Giacomo R.; Kroopnick, Peter M. (1984). "Primary productivity and particle fluxes on a transect of the equator at 153°W in the Pacific Ocean". Deep Sea Research Part A. Oceanographic Research Papers. 31 (1): 1–11. Bibcode:1984DSRA...31....1B. doi:10.1016/0198-0149(84)90068-2.
- ↑ Berger, W. H., Fischer, K., Lai, C., and Wu, G. (1987). "Ocean carbon flux: global maps of primary production and export production,". In: Biogeochemical Cycling and Fluxes between the Deep Euphotic Zone and Other Oceanic Realms", Vol. 3, ed. C. Agegian (Silver Spring, MD: NOAA), pages 87–30.
- ↑ Pace, Michael L.; Knauer, George A.; Karl, David M.; Martin, John H. (1987). "Primary production, new production and vertical flux in the eastern Pacific Ocean". Nature. 325 (6107): 803–804. Bibcode:1987Natur.325..803P. doi:10.1038/325803a0. S2CID 4353764.
- 1 2 3 Martin, John H.; Knauer, George A.; Karl, David M.; Broenkow, William W. (1987). "VERTEX: Carbon cycling in the northeast Pacific". Deep Sea Research Part A. Oceanographic Research Papers. 34 (2): 267–285. Bibcode:1987DSRA...34..267M. doi:10.1016/0198-0149(87)90086-0.
- 1 2 Gloege, Lucas; McKinley, Galen A.; Mouw, Colleen B.; Ciochetto, Audrey B. (2017). "Global evaluation of particulate organic carbon flux parameterizations and implications for atmospheric pCO2". Global Biogeochemical Cycles. 31 (7): 1192–1215. Bibcode:2017GBioC..31.1192G. doi:10.1002/2016GB005535. S2CID 133746237.
- 1 2 3 Cael, B. B.; Bisson, Kelsey (2018). "Particle Flux Parameterizations: Quantitative and Mechanistic Similarities and Differences". Frontiers in Marine Science. 5. doi:10.3389/fmars.2018.00395. hdl:1912/23677.
- ↑ Berelson, W. M. (2001). "The flux of particulate organic carbon into the ocean interior.: a comparison of four U.S. JGOFS regional studies". Oceanography, 14: 59–64.
- ↑ Yamanaka, Yasuhiro; Tajika, Eiichi (1996). "The role of the vertical fluxes of particulate organic matter and calcite in the oceanic carbon cycle: Studies using an ocean biogeochemical general circulation model". Global Biogeochemical Cycles. 10 (2): 361–382. Bibcode:1996GBioC..10..361Y. doi:10.1029/96gb00634.
- ↑ Kwon, Eun Young; Primeau, François; Sarmiento, Jorge L. (2009). "The impact of remineralization depth on the air–sea carbon balance". Nature Geoscience. 2 (9): 630–635. Bibcode:2009NatGe...2..630K. doi:10.1038/ngeo612. S2CID 59058813.
- ↑ Wilson, Jamie D.; Barker, Stephen; Edwards, Neil R.; Holden, Philip B.; Ridgwell, Andy (2019). "Sensitivity of atmospheric CO2 to regional variability in particulate organic matter remineralization depths". Biogeosciences. 16 (14): 2923–2936. Bibcode:2019BGeo...16.2923W. doi:10.5194/bg-16-2923-2019. S2CID 202987265.
- 1 2 Armstrong, Robert A.; Lee, Cindy; Hedges, John I.; Honjo, Susumu; Wakeham, Stuart G. (2001). "A new, mechanistic model for organic carbon fluxes in the ocean based on the quantitative association of POC with ballast minerals". Deep-Sea Research Part II: Topical Studies in Oceanography. 49 (1–3): 219–236. Bibcode:2001DSRII..49..219A. doi:10.1016/S0967-0645(01)00101-1.
- 1 2 Banse, Karl (1990). "New views on the degradation and disposition of organic particles as collected by sediment traps in the open sea". Deep Sea Research Part A. Oceanographic Research Papers. 37 (7): 1177–1195. Bibcode:1990DSRA...37.1177B. doi:10.1016/0198-0149(90)90058-4.
- ↑ Kriest, I.; Oschlies, A. (2008). "On the treatment of particulate organic matter sinking in large-scale models of marine biogeochemical cycles". Biogeosciences. 5 (1): 55–72. Bibcode:2008BGeo....5...55K. doi:10.5194/bg-5-55-2008. S2CID 3840184.
- ↑ Lutz, Michael; Dunbar, Robert; Caldeira, Ken (2002). "Regional variability in the vertical flux of particulate organic carbon in the ocean interior". Global Biogeochemical Cycles. 16 (3): 11-1–11-18. Bibcode:2002GBioC..16.1037L. doi:10.1029/2000GB001383.
- ↑ Middelburg, Jack J. (1989). "A simple rate model for organic matter decomposition in marine sediments". Geochimica et Cosmochimica Acta. 53 (7): 1577–1581. Bibcode:1989GeCoA..53.1577M. doi:10.1016/0016-7037(89)90239-1.
- ↑ Schwinger, Jörg; Goris, Nadine; Tjiputra, Jerry F.; Kriest, Iris; Bentsen, Mats; Bethke, Ingo; Ilicak, Mehmet; Assmann, Karen M.; Heinze, Christoph (2016). "Evaluation of NorESM-OC (Versions 1 and 1.2), the ocean carbon-cycle stand-alone configuration of the Norwegian Earth System Model (NorESM1)". Geoscientific Model Development. 9 (8): 2589–2622. Bibcode:2016GMD.....9.2589S. doi:10.5194/gmd-9-2589-2016. S2CID 59034696.
- ↑ Kriest, I.; Oschlies, A. (2011). "Numerical effects on organic-matter sedimentation and remineralization in biogeochemical ocean models". Ocean Modelling. 39 (3–4): 275–283. Bibcode:2011OcMod..39..275K. doi:10.1016/j.ocemod.2011.05.001.
- 1 2 3 4 Lauderdale, Jonathan Maitland; Cael, B. B. (2021). "Impact of Remineralization Profile Shape on the Air‐Sea Carbon Balance". Geophysical Research Letters. 48 (7): e2020GL091746. Bibcode:2021GeoRL..4891746L. doi:10.1029/2020GL091746. hdl:1721.1/130486. PMC 8243937. PMID 34219838.
Material was copied from this source, which is available under a Creative Commons Attribution 4.0 International License.
- ↑ Buesseler, Ken O.; Boyd, Philip W.; Black, Erin E.; Siegel, David A. (2020). "Metrics that matter for assessing the ocean biological carbon pump". Proceedings of the National Academy of Sciences. 117 (18): 9679–9687. Bibcode:2020PNAS..117.9679B. doi:10.1073/pnas.1918114117. PMC 7211944. PMID 32253312.
- ↑ Ittekkot, Venugopalan (1993). "The abiotically driven biological pump in the ocean and short-term fluctuations in atmospheric CO2 contents". Global and Planetary Change. 8 (1–2): 17–25. Bibcode:1993GPC.....8...17I. doi:10.1016/0921-8181(93)90060-2.
- ↑ Klaas, Christine; Archer, David E. (2002). "Association of sinking organic matter with various types of mineral ballast in the deep sea: Implications for the rain ratio". Global Biogeochemical Cycles. 16 (4): 63-1–63-14. Bibcode:2002GBioC..16.1116K. doi:10.1029/2001GB001765.
- 1 2 Francois, Roger; Honjo, Susumu; Krishfield, Richard; Manganini, Steve (2002). "Factors controlling the flux of organic carbon to the bathypelagic zone of the ocean". Global Biogeochemical Cycles. 16 (4): 34-1–34-20. Bibcode:2002GBioC..16.1087F. doi:10.1029/2001GB001722. S2CID 128876389.
- ↑ Fischer, G.; Karakaş, G. (2009). "Sinking rates and ballast composition of particles in the Atlantic Ocean: Implications for the organic carbon fluxes to the deep ocean". Biogeosciences. 6 (1): 85–102. Bibcode:2009BGeo....6...85F. doi:10.5194/bg-6-85-2009. S2CID 45117580.
- ↑ Sukigara, Chiho; Mino, Yoshihisa; Kawakami, Hajime; Honda, Makio C.; Fujiki, Tetsuichi; Matsumoto, Kazuhiko; Wakita, Masahide; Saino, Toshiro (2019). "Sinking dynamics of particulate matter in the subarctic and subtropical regions of the western North Pacific". Deep Sea Research Part I: Oceanographic Research Papers. 144: 17–27. Bibcode:2019DSRI..144...17S. doi:10.1016/j.dsr.2018.11.004. S2CID 133654627.
- ↑ Heinze, C. (2004). "Simulating oceanic CaCO3export production in the greenhouse". Geophysical Research Letters. 31 (16). Bibcode:2004GeoRL..3116308H. doi:10.1029/2004GL020613.
- ↑ Laws, Edward A.; Falkowski, Paul G.; Smith, Walker O.; Ducklow, Hugh; McCarthy, James J. (2000). "Temperature effects on export production in the open ocean". Global Biogeochemical Cycles. 14 (4): 1231–1246. Bibcode:2000GBioC..14.1231L. doi:10.1029/1999gb001229. S2CID 53998465.
- 1 2 3 4 Henson, Stephanie A.; Sanders, Richard; Madsen, Esben (2012). "Global patterns in efficiency of particulate organic carbon export and transfer to the deep ocean". Global Biogeochemical Cycles. 26 (1): n/a. Bibcode:2012GBioC..26.1028H. doi:10.1029/2011GB004099.
- ↑ Maerz, Joeran; Six, Katharina D.; Stemmler, Irene; Ahmerkamp, Soeren; Ilyina, Tatiana (2020). "Microstructure and composition of marine aggregates as co-determinants for vertical particulate organic carbon transfer in the global ocean". Biogeosciences. 17 (7): 1765–1803. Bibcode:2020BGeo...17.1765M. doi:10.5194/bg-17-1765-2020. hdl:21.11116/0000-0004-BD3E-3. S2CID 216206324.
- ↑ Buesseler, Ken O. (1998). "The decoupling of production and particulate export in the surface ocean". Global Biogeochemical Cycles. 12 (2): 297–310. Bibcode:1998GBioC..12..297B. doi:10.1029/97GB03366.
- ↑ Buesseler, K. O.; Lamborg, C. H.; Boyd, P. W.; Lam, P. J.; Trull, T. W.; Bidigare, R. R.; Bishop, J. K. B.; Casciotti, K. L.; Dehairs, F.; Elskens, M.; Honda, M.; Karl, D. M.; Siegel, D. A.; Silver, M. W.; Steinberg, D. K.; Valdes, J.; Van Mooy, B.; Wilson, S. (2007). "Revisiting Carbon Flux Through the Ocean's Twilight Zone". Science. 316 (5824): 567–570. Bibcode:2007Sci...316..567B. doi:10.1126/science.1137959. PMID 17463282. S2CID 8423647.
- ↑ Buesseler, K.O.; Trull, T.W.; Steinberg, D.K.; Silver, M.W.; Siegel, D.A.; Saitoh, S.-I.; Lamborg, C.H.; Lam, P.J.; Karl, D.M.; Jiao, N.Z.; Honda, M.C.; Elskens, M.; Dehairs, F.; Brown, S.L.; Boyd, P.W.; Bishop, J.K.B.; Bidigare, R.R. (2008). "VERTIGO (VERtical Transport in the Global Ocean): A study of particle sources and flux attenuation in the North Pacific". Deep-Sea Research Part II: Topical Studies in Oceanography. 55 (14–15): 1522–1539. Bibcode:2008DSRII..55.1522B. doi:10.1016/j.dsr2.2008.04.024.
- ↑ Honda, Makio C.; Watanabe, Shuichi (2010). "Importance of biogenic opal as ballast of particulate organic carbon (POC) transport and existence of mineral ballast-associated and residual POC in the Western Pacific Subarctic Gyre". Geophysical Research Letters. 37 (2): n/a. Bibcode:2010GeoRL..37.2605H. doi:10.1029/2009GL041521.
- 1 2 Weber, Thomas; Cram, Jacob A.; Leung, Shirley W.; Devries, Timothy; Deutsch, Curtis (2016). "Deep ocean nutrients imply large latitudinal variation in particle transfer efficiency". Proceedings of the National Academy of Sciences. 113 (31): 8606–8611. Bibcode:2016PNAS..113.8606W. doi:10.1073/pnas.1604414113. PMC 4978250. PMID 27457946.
- 1 2 Bach, L. T.; Stange, P.; Taucher, J.; Achterberg, E. P.; Algueró‐Muñiz, M.; Horn, H.; Esposito, M.; Riebesell, U. (2019). "The Influence of Plankton Community Structure on Sinking Velocity and Remineralization Rate of Marine Aggregates". Global Biogeochemical Cycles. 33 (8): 971–994. Bibcode:2019GBioC..33..971B. doi:10.1029/2019GB006256. S2CID 201329875.
- 1 2 3 4 5 6 Marsay, Chris M.; Sanders, Richard J.; Henson, Stephanie A.; Pabortsava, Katsiaryna; Achterberg, Eric P.; Lampitt, Richard S. (2015). "Attenuation of sinking particulate organic carbon flux through the mesopelagic ocean". Proceedings of the National Academy of Sciences. 112 (4): 1089–1094. Bibcode:2015PNAS..112.1089M. doi:10.1073/pnas.1415311112. PMC 4313834. PMID 25561526.
- 1 2 3 Devries, Tim; Weber, Thomas (2017). "The export and fate of organic matter in the ocean: New constraints from combining satellite and oceanographic tracer observations". Global Biogeochemical Cycles. 31 (3): 535–555. Bibcode:2017GBioC..31..535D. doi:10.1002/2016GB005551. S2CID 132192896.
- ↑ Laufkötter, C.; John, Jasmin G.; Stock, Charles A.; Dunne, John P. (2017). "Temperature and oxygen dependence of the remineralization of organic matter". Global Biogeochemical Cycles. 31 (7): 1038–1050. Bibcode:2017GBioC..31.1038L. doi:10.1002/2017GB005643. S2CID 102953187.
- 1 2 3 Pavia, Frank J.; Anderson, Robert F.; Lam, Phoebe J.; Cael, B. B.; Vivancos, Sebastian M.; Fleisher, Martin Q.; Lu, Yanbin; Zhang, Pu; Cheng, Hai; Edwards, R. Lawrence (2019). "Shallow particulate organic carbon regeneration in the South Pacific Ocean". Proceedings of the National Academy of Sciences. 116 (20): 9753–9758. Bibcode:2019PNAS..116.9753P. doi:10.1073/pnas.1901863116. PMC 6525517. PMID 31036647.
- ↑ McDonnell, A. M. P.; Boyd, P. W.; Buesseler, K. O. (2015). "Effects of sinking velocities and microbial respiration rates on the attenuation of particulate carbon fluxes through the mesopelagic zone". Global Biogeochemical Cycles. 29 (2): 175–193. Bibcode:2015GBioC..29..175M. doi:10.1002/2014GB004935. hdl:1912/7333. S2CID 53453555.
- 1 2 Henson, Stephanie; Le Moigne, Fred; Giering, Sarah (2019). "Drivers of Carbon Export Efficiency in the Global Ocean". Global Biogeochemical Cycles. 33 (7): 891–903. Bibcode:2019GBioC..33..891H. doi:10.1029/2018GB006158. PMC 7006809. PMID 32063666.
- ↑ Smith, Kenneth L.; Ruhl, Henry A.; Huffard, Christine L.; Messié, Monique; Kahru, Mati (2018). "Episodic organic carbon fluxes from surface ocean to abyssal depths during long-term monitoring in NE Pacific". Proceedings of the National Academy of Sciences. 115 (48): 12235–12240. Bibcode:2018PNAS..11512235S. doi:10.1073/pnas.1814559115. PMC 6275536. PMID 30429327.
- ↑ New Research Reveals Uncertainty in How Much Carbon the Ocean Absorbs Over Time – Climate Projections Could Be Off SciTechDaily, 8 May 2021.
- ↑ Ito, Takamitsu; Follows, Michael J. (2005). "Preformed phosphate, soft tissue pump and atmospheric CO2". Journal of Marine Research. 63 (4): 813–839. doi:10.1357/0022240054663231.
- ↑ Volk, Tyler; Hoffert, Martin I. (2013). "Ocean Carbon Pumps: Analysis of Relative Strengths and Efficiencies in Ocean-Driven Atmospheric CO2 Changes". The Carbon Cycle and Atmospheric CO2 : Natural Variations Archean to Present. Geophysical Monograph Series. pp. 99–110. doi:10.1029/GM032p0099. ISBN 9781118664322.
- ↑ Boyd, Philip W.; Claustre, Hervé; Levy, Marina; Siegel, David A.; Weber, Thomas (2019). "Multi-faceted particle pumps drive carbon sequestration in the ocean" (PDF). Nature. 568 (7752): 327–335. Bibcode:2019Natur.568..327B. doi:10.1038/s41586-019-1098-2. PMID 30996317. S2CID 119513489.